|
 |
RECEPTORS
The central
nervous system is kept continually informed of the ever-changing
external and internal environment of the body by way of
centrally directed signals which arise in its many and varied
receptors. These receptors report on a wide variety of sensory
modalities including changes in temperature, pressure, touch,
sound, light, taste, smell, body and limb movements, and even
blood pressure and chemistry. Scientists have recognized for
almost 130 years that certain afferent nerve fibers of the
peripheral nervous system are in contact with specialized non
neural receptive structures which detect and transmit sensory
information from the periphery to the CNS. The nonneural
receptive structure together with its afferent nerve fiber is
often called a receptor.
Nature has
evolved a variety of morphological structures which function as
receptors. The earliest studies of sensation led to the idea
that each morphological receptor type was responsible for the
transduction of a particular modality of sensation. This early
hypothesis has been modified in light of evidence that receptors
respond to more than one type of stimuli.
CLASSIFICATION OF RECEPTORS BY ADEQUATE
STIMULI
An adequate
stimulus is that form of stimulation to which a receptor has the
lowest threshold. For example, a certain type of receptor will
respond to a slight mechanical displacement by increasing the
impulse firing rate in its afferent nerve fiber. The same
receptor may also respond when subjected to extreme temperature
changes. However, if it has a lower threshold for mechanical
than for thermal changes, it is classified as a mechanoreceptor
and not a thermoreceptor. Accordingly, receptors are often
classified as follows:
Receptor type |
Adequate stimulus
|
Mechanoreceptors |
Mechanical displacement
|
Thermoreceptors |
Temperature change
|
Nociceptors |
Pain |
Chemoreceptors |
Chemicals |
Photoreceptors |
Light |
Recognize
that this classification does not mean that the adequate
stimulus is the only stimulus to which a particular receptor
will respond. It simply says that the receptor has the lowest
threshold for (is most easily simulated by) the adequate
stimulus.
Mechanoreceptors, thermoreceptors, and nociceptors in
cutaneous, subcutaneous, and deep connective tissue are
collectively called somatosensory receptors. While the
morphological endings of many of these are unknown, the
remainder are classified as either free endings, endings
with expanded tips, or encapsulated endings (Fig-1).
Free nerve endings represent receptors with no nonneural
element. Instead, the afferent fibers simply end in bare
terminals which are directly susceptible to stimulation.
Similarly, endings with expanded tips such as Merkel's
disks and Ruffini endings are all neural structures
which respond directly to adequate stimulation. However,
receptors with encapsulated endings are characterized by
a nonneural element surrounding the afferent endings of
the nerve fibers. In receptors of this type the adequate
stimulus must first be transduced through the nonneural
capsule to the endings of the afferent nerve fiber. |
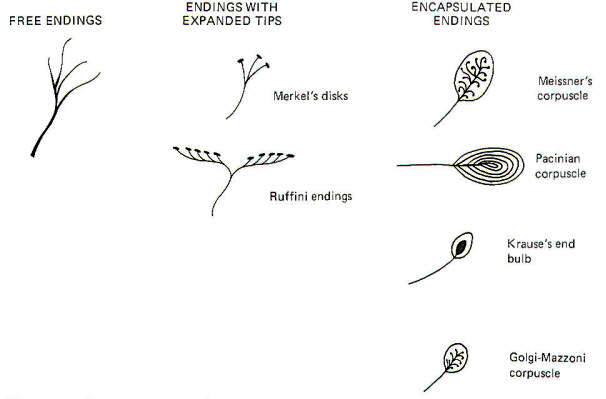 |
Fig-1 |
THE NATURE OF THE RECEPTOR POTENTIAL
When a
stimulus is applied to a receptor, it may or may not be strong
enough to elicit impulse production in the afferent nerve fiber.
The application of the stimulus causes the membrane of the
receptor cell to depolarize, producing a receptor potential
(RP). If the receptor potential reaches the excitation threshold
of the nerve fiber membrane, the fiber will generate impulses.
Further, as long as the receptor potential is maintained above
the excitation threshold, impulses will continue to travel down
the fiber away from the receptive element. A distinction can be
made between receptors in which the receptive element is a
specialized ending of the nerve fiber sharing a continuous
membrane and receptors in which the receptive element is a
separate structure not continuous with the membrane of the nerve
fiber. In the former (one-element) receptor, the RP established
in the receptive element produces impulses in the adjacent
membrane by depolarizing this membrane with electrotonic
currents. In the latter (twoelement) receptor the RP is
generated in the separate receptive element, which in turn
stimulates and produces impulses in the afferent nerve fiber.
The mechanism by which a RP in the separate receptive element
does this is not well understood. It may be that the close
proximity of the two allows for a current spread between them
or, as is suspected in some cases, a chemical transmitter may be
released from the receptive element to the afferent nerve fiber.
When not
being stimulated, the membrane potential of the receptor is
resting and polarized. However, when a stimulus is applied and
its strength is steadily increased, the receptor membrane begins
to depolarize and a RP is established (Fig-2).
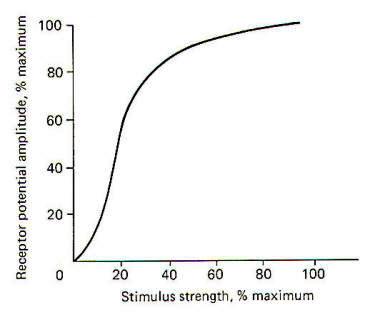 |
Fig-2 |
It is
thought that the receptor potential is produced by changes in
the ionic current across the membrane of the receptive element.
The depolarization phase of the receptor potential is very
likely caused by the inward diffusion of Na+ ions.
Repolarization is less well understood but is probably caused by
ionic changes also. The receptor potential increases as a
function of the stimulus strength and is therefore graded.
However, it must be understood that Fig-2, which shows the
relationship between the two, is based on a pacinian corpuscle
from the cat mesentery and does not represent all types of
receptors. Mathematical attempts have been made to predict the
receptor potential from the strength of the stimulus but have
thus far been inaccurate when applied to different types of
receptors.
Receptor Potentials and Impulse
Generation
It
is important to understand that in an afferent neuron
which conducts impulses following stimulation of its
receptive element, the impulses are not generated in the
receptive element itself. Instead, they are initiated at
some point central to the receptor. Only the receptor
potential is initiated in the receptive element. In
one-element receptors like the pacinian corpuscle
illustrated in Fig-3, the trigger for the production of
impulses is the spread of an electrotonic current from
the receptive element to the "active zone" of the nerve
fiber just central to the receptor.
When a slight mechanical displacement is applied to the
corpuscle of the receptor, changes in ionic conduction
occur in the membrane of the afferent fiber within it,
depolarizing its membrane and producing a small receptor
potential. The RP generates a small electrotonic current
which spreads a short distance down the fiber central to
the point of stimulation. No impulses are recorded in
the afferent fiber, however, as the electrotonic current
is too small to reach and subsequently depolarize the
"active zone" (first node of Ranvier). However, as the
strength of the applied stimulus is systematically
increased, the size of the RP and thus the electrotonic
current increases also. When the current is sufficiently
strong to not only reach but also depolarize the
membrane of the first node to the excitation threshold,
an action potential is generated at the node which
propagates by ordinary saltatory conduction down the
length of the fiber. Further, the first node continues
to produce action potentials and generate impulses as
long as the membrane of the first node remains above the
excitation threshold. Notice that impulses are not
generated in the same region of the receptor that
produces the receptor potential. Thus it is commonly
said that the receptor potential is a graded but
nonpropagated event, while the action potential is
nongraded but propagated. Nerve fibers continue to
conduct impulses as long as the stimulus is applied and
the excitation threshold of the active zone is exceeded.
The firing rate depends on the magnitude of the receptor
potential (Fig-4), which itself depends on the strength
of the applied stimulus. |
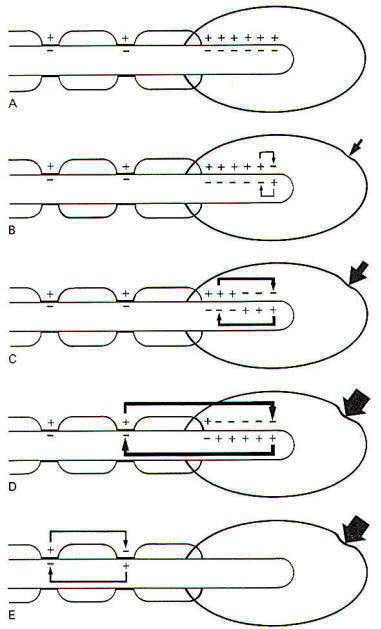 |
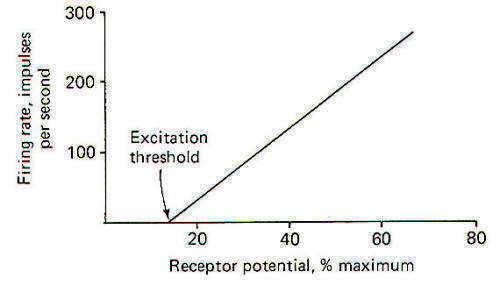 |
Fig-3 |
Fig-4 |
Adaptation by Receptors
When a receptor is strongly stimulated a high initial
firing rate is established in the nerve fiber which
decreases somewhat with time. Even when the stimulus is
continually applied with the same intensity, the firing
rate decreases within a few seconds. This decrease in
the firing rate in spite of constant stimulation is
called adaptation. All receptors adapt to some extent
with the possible exception of pain receptors. Certain
receptors (i.e., hair receptors and pacinian corpuscles)
adapt very quickly and are referred to as rapidly
adapting receptors. As you can see in Fig-5, their
firing rates drop to zero within a second or two even in
the face of constant stimulation. In other words their
receptor potentials decreased below the excitation
threshold and impulse conduction stopped. Other
receptors (i.e., muscle spindles) adapt much more slowly
and even then only to a limited degree. Their firing
rates usually level off to a steady, although lower rate
than initially recorded. These are slowly adapting
receptors. The receptor potential also decreases here,
but generally not below the excitation threshold for
impulse firing. It is apparent that rapidly adapting
receptors are particularly adept at signaling the
presence of a stimulus only at the outset of
stimulation. Consequently they are classed as phasic
receptors. On the other hand, slowly adapting receptors
continually signal the presence of a stimulus and are
often referred to as tonic receptors. |
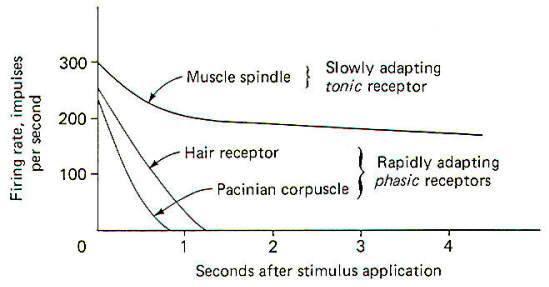 |
Fig-5 |
MECHANORECEPTORS: A CLOSER EXAMINATION
Mechanoreceptors by definition respond to mechanical
displacement. Pushing the skin on the back of one hand
with the finger of the other hand, for instance,
displaces a great number of cutaneous mechanoreceptors.
Similarly, joint receptors respond to mechanical
displacement during movement of a limb. While the body
has many individual examples of mechanoreceptors, they
can be conveniently grouped into three broad categories
(Fig-6). Position and velocity mechanoreceptors respond
by firing impulses when the stimulating source is
stationary as well as when it is moving. Velocity
mechanoreceptors, on the other hand, fire only when the
stimulating source is moving and stop or become "silent"
once the mechanoreceptor has been displaced to a new
fixed position. The third group, transient
mechanoreceptors, fire only at the onset of a
displacement.
While some mechanoreceptors fall in only one of the
three groups, it is important to recognize that others
show characteristics of two or even all three of the
groups. There appear to be no receptors which respond
strictly to position. Nevertheless, it is likely that
all position receptors show some degree of velocity
response.
When a mechanoreceptor is stimulated, its firing rate
increases. As the degree of displacement increases so
does the firing rate. At a certain level of displacement
the firing rate stops increasing even in the face of
continual displacement (Fig-7). The firing rate at the
greatest displacement minus the firing rate at the least
displacement represents the dynamic range of the
receptor. |
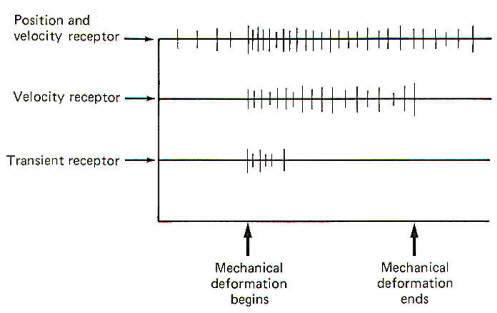 |
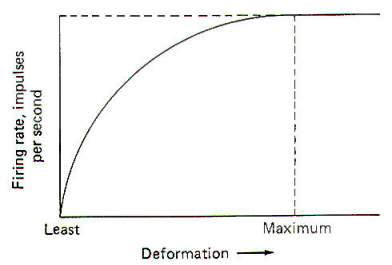 |
Fig-6 |
Fig-7 |
Mechanoreceptors in Hairy Skin
All three
types of mechanoreceptors are found in hairy skin. While these
three types are also found in glabrous (hairless) skin, there
are significant differences between individual receptors.
Position and Velocity Receptors
Two types
of position and velocity receptors are found in hairy skin. Type
I receptors are the peripheral ends of type A beta fibers
associated with Merkel's disks. They are stimulated by
indentation of the skin and respond with an irregular discharge.
They show a good velocity response but a smaller position
response. Type II receptors are the peripheral ends of type A
beta fibers which terminate in Ruffini corpuscles. They are
stimulated by deformation of the skin and respond with a regular
discharge. Unlike type I receptors, they have a good position
response but a smaller velocity response. Both type I and type
II receptors are slowly adapting and are thus able to give rise
to conscious sensation associated with both instantaneous and
prolonged skin displacement.
Velocity Receptors
Four types
of velocity receptors are found in hairy skin. G2 hair receptors
are the peripheral ends of type A beta fibers terminating around
the base of guard hairs in the base of hair follicles. They
respond to both slow and rapid movement of hairs and deflection
of the skin. Field receptors are associated with type A beta
fibers and their terminal morphology is unknown. They respond to
indentation of the skin. D hair receptors are the terminal
endings of type A delta fibers terminating around the base of
both guard and down (fine) hair. They respond to both slow and
rapid movements of these hairs as well as to skin deflection. C
mechanoreceptors are rare, typically being associated with
nonmyelinated type C fibers. Their terminal morphology is
unknown and they respond only to slow displacement of the skin.
Transient Receptors
Two types
of transient receptors are found in hairy skin. Pacinian
corpuscle receptors are associated with the peripheral ends of
certain type A alpha and beta fibers. They respond to mechanical
"taps" and vibrations in the 50- to 500-Hz range. G 1 hair
receptors are specialized processes at the base of hair
follicles associated with large type A alpha fibers and they
respond to high-velocity guard hair and skin displacement.
Mechanoreceptors in Glabrous
(Hairless) Skin
Like hairy
skin, glabrous skin also contains position and velocity,
velocity, and transient receptors. Nevertheless, there are some
morphological differences between them such as the type of
afferent nerve fiber which carries the signal and the nature of
the receptive element itself.
Position and Velocity Receptors
The
position and velocity receptors in glabrous skin are classified
as slowly adapting (SA) receptors. It is likely that there is
more than one type present. Nevertheless, SA receptors are
associated with type A beta fibers and terminate in Ruffini-type
corpuscles and possibly Merkel's disks. They respond to
indentation of the skin.
Velocity
Receptors
Velocity receptors in glabrous skin are classified as
rapidly adapting (RA) receptors. RA receptors are associated
with type A alpha fibers and possibly terminate in Meissner's
corpuscles. Like SA receptors they respond to skin indentation.
Transient Receptors
The transient receptors in glabrous skin are
also pacinian corpuscles. They have the same morphological and
stimulating characteristics as those in glabrous skin.
Mechanoreceptors in Muscles and Tendons
Strict
velocity receptors do not appear to be present in this group.
However, transient receptors and several kinds of position and
velocity receptors have been identified.
Position and Velocity
Receptors
The position and velocity receptors in this group
include the muscle spindles, Golgi tendon organs, and pressure
receptors. Muscle spindles are associated with both group Ia and
group II nerve fibers and respond both to change and rate of
change in muscle length. Golgi tendon organs are the terminal
endings of group Ib fibers and they respond to the tension
developed in fascia and contracting or stretched muscle insofar
as it applies tension to tendons. Pressure receptors respond to
pressure on the belly of the muscle primarily, and to any
distortion of the fascia surrounding the muscle. They are
associated with certain group III fibers and their terminal
morphology is unknown.
Transient
Receptors
Transient receptors are again of the pacinian
corpuscle type. They are associated with group II fibers and
respond to both "taps" and vibrations in the 50- to 500-Hz
range.
Mechanoreceptors in Joints
All three
types of mechanoreceptors are represented in joints. However,
their distribution is not uniform.
Position
and Velocity Receptors
Position and velocity receptors are the
most abundant type of mechanoreceptors found in joints. They
fall into two categories. SA type 1 receptors are associated
with myelinated fibers greater than 10
µm in diameter which
terminate in Golgi-type organs. They are located in the joint
ligaments and respond both to joint position and movement. SA
type 2 receptors terminate in Ruffini-type endings and are
associated with type A beta fibers. They respond to joint
bending and discharge in the absence of movement to give
position sense and during movement to give velocity sense.
Velocity
Receptors
The velocity receptors signal phasic stimuli. Their
terminal morphology is unknown but they are associated with
type A alpha fibers and respond to joint movement, particularly
of a bending and twisting nature.
Transient
Receptors
This group represents the least common joint receptor
responding to mechanical transients in joint movement. They
signal "tap" stimuli and are associated with type A alpha fibers
which terminate in paciniantype corpuscles. They discharge
whenever the joint is moved regardless of the direction, and
their response is brief.
Mechanoreceptors in Special Sense Organs
The ear and
the vestibular system make interesting use of mechanoreceptors.
Organ of Corti hair cells respond to sound-induced movements of
the basilar membrane of the inner ear. Special somatic afferent
(SSA) fibers of cranial nerve VIII are stimulated when the hairs
are bent. Vestibular system hair cells, located in the crista
ampullaris and macula acustica of the vestibular apparatus,
respond to angular movements, linear acceleration, and the
position of the head in space. SSA fibers of cranial nerve VIII
located at the base of the hair cells respond when the hairs are
bent, pushed, or pulled.
Mechanoreceptors in the Viscera
A number of
mechanoreceptors operate in the visceral organs and blood
vessels. Carotid sinus and aortic baroreceptors, located in the
walls of the carotid sinus and the aorta, respectively, respond
to changes in blood pressure. Their terminal morphology is
unknown, but general visceral afferent (GVA) fibers of cranial
nerves IX (glossopharyngeal) and X (vagus), respectively,
connect the receptive elements with the brainstem. Alveolar
stretch receptors located in the walls of the pulmonary alveoli
are the peripheral endings of GVA fibers of the vagus nerve.
They respond to inflation and deflation of the lungs and their
terminal morphology is unknown.
Gastrointestinal (GI)
stretch receptors are located throughout the
walls of the GI tube from the pharynx to the rectum. They
respond to stretch of the tube and subsequently conduct impulses
to the CNS over GVA fibers of cranial nerves V (trigeminal),
IX, and X as well as certain afferent fibers of the pelvic
nerves. Urinary bladder stretch receptors are located in the
walls of the detrusor muscle of the bladder. Their terminal
receptive elements are associated with the GVA fibers of the
pelvic nerve and they respond to filling of the bladder.
THERMORECEPTORS
Thermoreceptors respond to changes in temperature. Little is
know about visceral temperature receptors and consequently most
of our knowledge is limited to cutaneous thermoreceptors. Strict
thermoreceptors (those with a lower threshold to thermal changes
than to mechanical or noxious stimuli) are classified as warm
or cold receptors. Warm receptors respond to temperature
increases of greater than 0.1oC in the range from 30 to 43°C.
Cold receptors respond to temperature decreases of greater than
0.1oC in the range from 35 to 15°C (Fig-8). It is likely
that the brain has learned to interpret the relative ratio of
warm and cold receptor firing as indicative of a particular
temperature in the region where the response of the two
receptors overlap. |
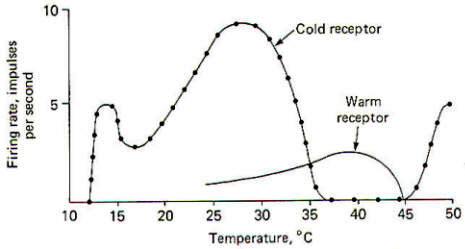 |
Fig-8 |
NOCICEPTORS
Receptors
which respond primarily to injurious or painful stimulation are
called nociceptors. Within this general category are four
subgroups: mechanonociceptors, mechano-heat nociceptors,
mechano-cold nociceptors, and poly modal nociceptors.
Nociceptors are found in skin, muscles, joints, and the viscera.
Nociceptors
in the Skin
Each of the
four subgroups of nociceptors is represented in cutaneous
tissue. While their terminal morphology is unknown, they are
distinguished by their response patterns. Cutaneous
mechanonociceptors are associated with type A delta fibers and
respond to high shearing force. Cutaneous mechano-heat
nociceptors respond to noxious levels of mechanical stimulation
and heat in excess of 43°C. They are associated with certain
myelinated type A delta fibers. On the other hand, cutaneous mechano-cold nociceptors are the terminal endings of certain
nonmyelinated type C fibers. They are particularly adept at
responding to noxious levels of mechanical stimulation and
temperatures below 10°. Polymodal nociceptors respond to noxious
levels of mechanical, heat, and chemical stimulation and
represent the terminal endings of certain nonmyelinated type C
fibers.
Nociceptors
in Muscles, Joints, and Viscera
Two types
of muscle nociceptors have been identified. Pressure nociceptors
respond to strong pressure and excessive muscle stretch. Their
terminal morphology is unknown and they are associated with
myelinated group III fibers. Group IV nociceptors respond to
strong pressure, temperature extremes, and anoxia. Their
receptive elements are associated with nonmyelinated group IV
fibers.
Little is
known about joint and visceral nociceptors. Joint nociceptors
are the peripheral ends of certain type A delta fibers. They
respond to joint overextension and their terminal structures
are unidentified. Pain receptors in the viscera are probably not
located in the parenchyma of the internal organs themselves, but
are found instead in the peritoneal surfaces, pleural membranes,
dura mater, and the walls of blood vessels.
CHEMORECEPTORS
Chemoreceptors are defined as those receptors which respond most
easily to chemical stimulation. External chemoreceptors include
taste cells and olfactory cells, which give rise to the
conscious sensations of taste and smell. Internal chemoreceptors respond to changes in circulating PCO2 PO2, and
pH. They do not give rise to conscious sensation. Included in
this category are the carotid body and aortic chemoreceptors and
those chemoreceptors in the respiratory and vasomotor centers
of the brainstem.
External
Chemoreceptors
Taste Cells
The taste cell is the chemically sensitive element for the sense
of taste. Taste cells cluster together in small units called
taste buds (Fig-9). The average taste bud contains 20 or so
taste cells. Children have the greatest number of functional
taste buds, and the number decreases with age so that the adult
has about 10,000 functional buds. Each taste cell is typically
columnar in shape and is characterized by numerous microvilli
which project to a narrow opening at the top of the bud called a
taste pore. The base of the taste cells are in close contact
with the special visceral afferent (SVA) fibers of cranial
nerves VII and IX.
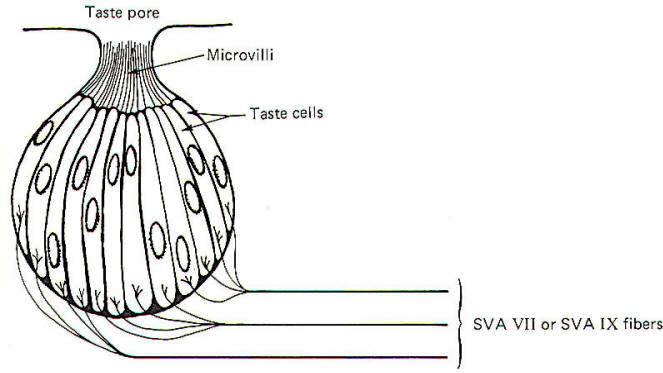 |
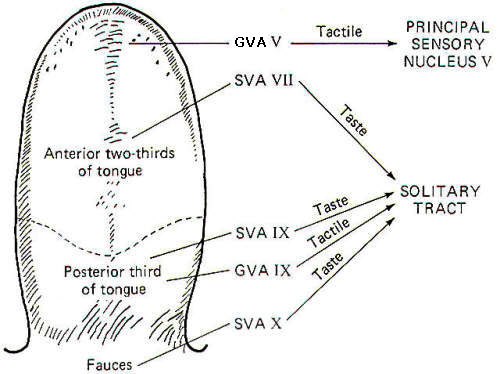 |
Fig-9 |
Fig-10 |
Papillae
Location
Taste buds are chiefly located in raised areas of the
tongue known as papillae. In addition, taste buds are located on
the epiglottis, the tonsilar pillars, and other areas of the
fauces (passage from mouth to pharynx). Numerous small fungiform
papillae are located over the anterior surface of the tongue.
These papillae contain a moderate number of buds, perhaps as
many as 100 per papilla. Much larger circumvallate papillae form
a V on the back of the tongue and contain up to 250 taste buds
each. Foliate papillae, located behind the circumvallate
papillae, contain fewer buds.
Afferent
Innervation of the Tongue and Fauces Fig-10 illustrates
that several afferent fibers conduct information from the
tongue. Touch (not taste) sensation
from the anterior two-thirds of the tongue is transmitted over GVA fibers of cranial nerve V to the principal sensory nucleus
of V in the pons, while tactile sensation from the posterior
one-third of the tongue is conducted over GVA fibers of
cranial nerve IX to the solitary tract of the medulla
oblongata.
Taste
sensation from the anterior two-thirds of the tongue is
transmitted over SVA fibers of cranial nerve VII, while SVA IX
fibers relay taste information from the posterior one-third. SVA X fibers conduct taste information from taste cells in the
fauces. All of these afferent taste-conducting pathways
terminate in the solitary tract.
Four Basic
Taste Modalities
Four basic taste modalities are generally
recognized. These are sweet, salty, sour, and bitter. Evidence
suggests that all taste buds respond to some degree to all four
stimuli. Nevertheless, buds on the tip of the tongue respond
most strongly to sweet and salty stimuli, while chemicals
giving rise to a sour sensation most effectively stimulate buds
along the edge. Chemicals associated with the bitter sensation
most effectively stimulate the base of the tongue.
The
adequate chemical stimuli for the four basic taste modalities
fall into characteristic chemical groups. For instance, the
chemicals which give rise to the sour sensation are usually
acids. The lower the pH , the more the taste cells are
stimulated. Sweet stimuli are usually organic molecules such as
sugars, glycols, aldehydes, and others. Alkaloids like quinine,
caffeine, and nicotine give rise to the bitter sensation, while
ionizable salts give rise to the sensation we describe as salty.
In order to
stimulate the taste cells within a taste bud, the stimulating
chemicals must dissolve in the saliva and then enter the taste
pore. Here they stimulate the taste cells, which in turn
stimulate the SVA endings of cranial nerves VII, IX, and X.
Adaptation of Taste Cell Chemoreceptors
When a taste stimulus is
first applied to the tongue, the sensation is strong and then
becomes weaker with time. The sourness becomes less sour, the
sweetness becomes less sweet. etc. I n other words, the taste
cells adapt to the stimulus. This subjective awareness of
decreasing sensation is paralleled by a decrease in the firing
rate of the SVA neurons (Fig-11).
Taste Discrimination
While
taste buds respond to the four basic taste stimuli, they do so
with different intensities. A particular taste bud may respond
with a high-frequency discharge to a sweet stimulus but produce
a lowfrequency discharge to salty, bitter, and sour stimuli. As
stated previously, those responding primarily to bitter stimuli
are concentrated at the base of the tongue, while those
responding with the greatest discharge frequency to sweet and
salty stimuli are concentrated at the tip. Sour receptors are
located along the edge. Fig-12 illustrates the different
sensitivities of two taste buds.
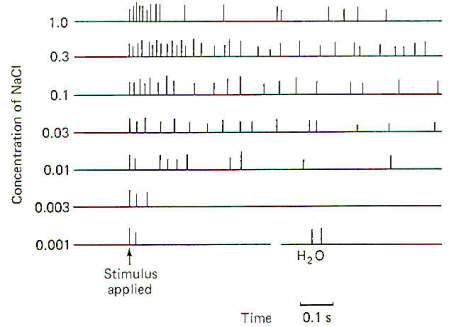 |
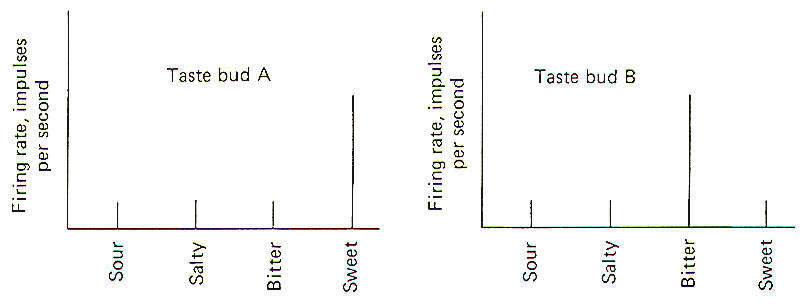 |
Fig-11 |
Fig-12 |
Taste bud A
is a "sweet" bud. That is, when a sweet stimulus is applied, a
much higher firing rate is initiated in the SVA fibers from its
taste cells than when a bitter, salty, or sour stimulus is
applied. Taste bud B, on the other hand, is a "bitter" bud
because it responds with the greatest discharge to bitter
stimuli. The brain probably interprets a given taste by
analysis of the discharge ratios of the different kinds of taste
buds stimulated. For example, if the firing rate from bud A is
10 times greater than from bud B when a chemical stimulus is
applied, the stimulus was probably quite sweet. On the other
hand, if the relative firing rates were reversed with bud B
responding with a firing rate 10 times greater than bud A, the
applied stimulus was probably quite bitter. Since the only
message a neuron can carry is an impulse, all of which are quite
similar, it follows that the only variable is the pattern of
firing (i.e., the rate, grouping patterns, etc.). Consequently
a possible partial explanation of how the conscious cortex
evaluates a given taste stimulus is by analysis of the relative
discharge patterns of the four basic kinds of taste buds from
each part of the tongue and fauces.
Such an integrated discharge pattern could supply the necessary
information to the brain to enable it to accurately sense even
the most subtle differences in taste.
Olfactory
Cells
The chemically receptive element for the sense of smell is
the olfactory cell. These cells, located in the olfactory mucosa
of the nasal cavity, project their peripheral processes into a
mucous layer which is exposed to the air in the nasal cavity.
Their central processes penetrate the cribiform plate of the
ethmoid bone to synapse with mitral cells in tufted olfactory
glomeruli (Fig-13).
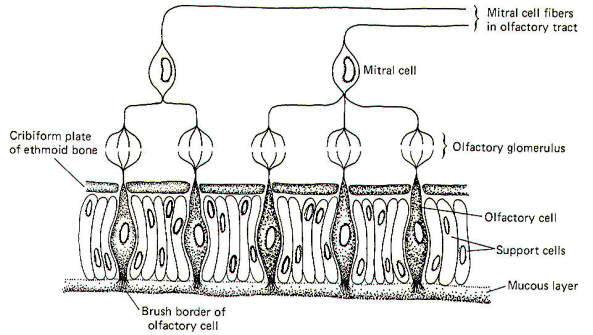 |
|
Fig-13 |
|
In addition
to olfactory cells, the olfactory mucosa is also made up of
support cells and mucus-secreting cells. The entire surface of
the olfactory mucosa occupies a little more than 5 cm2.
Conscious
and Reflex Olfactory Pathways
The axons of mitral cells pass
from the olfactory bulb centrally toward the brain as the
olfactory tract. The tract then divides to form separate medial
and lateral olfactory tracts. The lateral olfactory tract
ultimately terminates in the periamygdaloid cortex of the
temporal lobe. This pathway probably represents the conscious
smell pathway. The medial olfactory tract may terminate in the
septal nuclei, the contralateral amygdala, or the anterior
continuation of the hippocampus.
The body
reflexly responds to both pleasant and unpleasant odors. The
reflex responses are classified as viscerosomatic or
viscerovisceral, depending on the nature of the response.
Viscerosomatic reflexes include the reflex movements of the
eyes, facial muscles, neck and the rest of the body in response
to both pleasant and unpleasant odors. Viscerovisceral reflexes
include salivary and gastric secretions in response to certain
pleasant odors and vomiting in response to very obnoxious odors.
Both the medial and lateral olfactory tracts contribute to the
reflex pathways.
Odorants
Unlike
taste, no subjective classification of basic olfactory
modalities has been agreed upon. However, for any odorant to be
an effective stimuli it must be volatile. Water and lipid solubility are
also desirable qualities. Volatility is necessary to allow the
chemical to be adequately drawn into the nasal cavities, while
water solubility is necessary since the odorant must penetrate
the olfactory mucosa in order to reach the brush borders of the
olfactory cells. There is even some evidence that the odorant
must penetrate the brush border membrane in order to effectively
stimulate the olfactory cell, in which case lipid solubility
would be a desirable feature. In any event, the odorant
establishes a receptor potential in the olfactory cell, which
then gives rise to impulse production in the mitral cells of
the olfactory bulb. The mechanism of olfactory cell stimulation
of the mitral cells in unknown, but there is some evidence that
a chemical transmitter may be involved.
Olfactory
Discrimination
When an odorant of threshold concentration is
presented to the olfactory epithelium, the subject is barely
aware of its presence. If the concentration is increased, the
sensation increases as well. Finally, the sensation reaches a
maximum, and further increases in odorant concentration elicit
no further increases in sensation.
Allowing
for individual differences, maximum sensation is usually reached
with an odorant concentration 10 to 50 times greater than
threshold. This does not allow much dynamic range. It is
considerably less, for instance, than the range for vision
(about 500,000 to 1). It would appear that the olfactory system
is better designed for odor detection than for odor
quantification. Further support for the idea that odor
detection is perhaps the principal role of the olfactory system
is the adaptation which occurs in the face of a sustained
stimulus. The firing rate of olfactory tract neurons might
decrease by as much as 50 percent within the first second or
two following odorant application. This rapid decrease declines
after the first second or two, but the signal is very weak after
a minute or so.
Electroolfactogram
When an odorant is presented to the olfactory
epithelium a monophasic action potential called the
electroolfactogram (EOG) can be recorded. The amplitude of the
EOG is a function of the odorant concentration, and in all
probability represents the combined receptor potentials of many
olfactory cells. Receptor potential recordings from individual
olfactory cells has not yet been satisfactorily achieved.
Internal
Chemoreceptors
Internal
chemoreceptors include the carotid body and aortic
chemoreceptors and the chemically sensitive cells in the
respiratory and vasomotor centers of the
brainstem. The carotid body chemoreceptors have been subjected
to more study than the others partly because of their relative
accessibility. Recall that the internal chemoreceptors respond
to changes in circulating PCO2, PO2, and pH but do not give rise
to conscious sensation.
Functional
Arrangement of the Carotid Body Chemoreceptors
The carotid
bodies contain large glomus cells which make contact with the
endings of the GVA fibers of the glossopharyngeal nerve. Two
kinds of contacts are observed: small discrete bouton endings
to single glomus cells and large endings in contact with several
glomus cells (Fig-14).
|
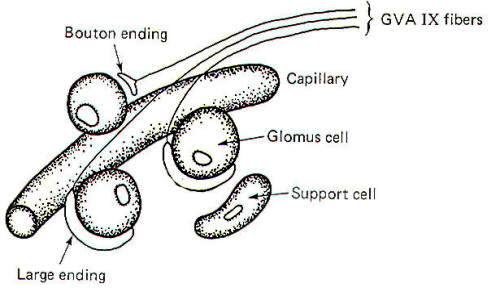 |
|
Fig-14 |
Carotid
Bodies Respond to Changes in PC02, P02, and pH The carotid
bodies are particularly suitable for blood chemistry testing as
about 20 ml per gram of carotid body tissue per minute is the
flow rate of blood through the carotid bodies in the cat. This
is among the highest tissue blood flow values found anywhere in
the body. The carotid bodies are particularly sensitive to
changes in the arterial oxygen concentration. When the P02 drops
below the normal level of about 95 mmHg, the GVA fibers from
the carotid bodies respond with an increase in their firing
rates. It is not presently known whether the GVA endings
themselves are directly stimulated by the oxygen drop or whether
the glomus cells are the chemosensitive elements which then
stimulate the bouton and large endings of the nerve fibers.
To a lesser
extent, the carotid bodies are also sensitive to changes in
blood PC02, and pH. Increasing the PC02 above the normal value of
40 mmHg or decreasing the arterial pH below the normal value of
7.4 produces increased firing in the GVA IX fibers. Because
of the close relationship between PC02 and pH it is difficult to
tell which event is the actual stimulus. Again, it is not known
whether the glomus cells of the endings of the afferent fibers
themselves are initially stimulated. There is some evidence that
chemical transmission is involved, however, and this would
point to the likelihood that the glomus cells themselves are the
actual receptive elements subsequently stimulating the afferent
endings of the GVA fibers by chemical transmission.
Evidence
for Cholinergic Transmission
If a chemical transmitter operates
in the carotid body chemoreceptor system, it is probably
acetylcholine. ACh is present in carotid body tissue. So are the
enzymes necessary for its synthesis (cholineacetyltransferase)
and degradation (acetylcholinesterase). In addition, carotid
bodies in vitro are sensitive to extremely small amounts of ACh,
and this sensitivity is enhanced by physostigmine (an
anticholinesterase). In vitro studies also show that the
response of the carotid bodies to natural stimulation is
decreased by the administration of curare and atropine.
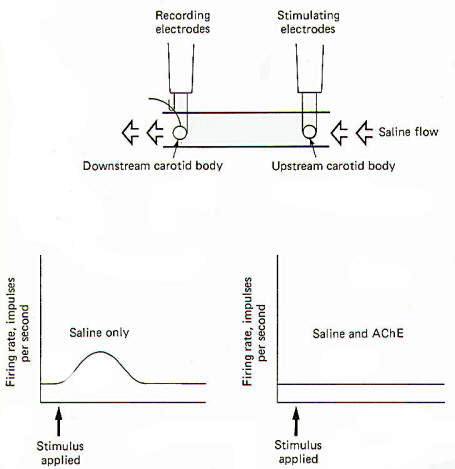 |
Fig-15 |
A technique
pioneered by Otto Loewi has been used to illustrate the
cholinergic nature of the carotid bodies. In Fig-15 two
carotid bodies, each with its
nervous innervation intact, are placed in a saline trough so
that physiological saline can flow freely over both of them in
a single direction. Stimulating electrodes are placed on the
upstream preparation and recording electrodes are placed on the
downstream preparation. When the two bodies are relatively close
to each other (9 mm), stimulation of the upstream preparation
produces, after an appropriate delay, increased firing in the
nerve of the downstream preparation. The implication is that a
chemical was released into the saline stream from the upstream
preparation which diffused downstream, subsequently stimulating
the downstream carotid body. Modification of this experiment
indicates the chemical may be ACh. If the same procedure is run
again with acetylcholinesterase (AChE) added to the saline flow,
no response is observed.
CLASSIFICATION OF RECEPTOR BY AFFERENT NERVE FIBER TYPE
The
receptors of the peripheral nervous system will be classified
(Table -1) according to the type of afferent nerve fiber
which conducts its signals to the central
nervous system. Receptors located within the brain (i.e.,
chemoreceptors of the hypothalamus and those in the respiratory
and vasomotor centers of the brainstem) are not included in this
classification system because they are not associated with
peripheral terminations of spinal and cranial afferent nerve
fibers.
Table-1
Classification of Receptor by Afferent Nerve Fiber Type |
I
|
General somatic
receptors. Respond to adequate stimulation of cutaneous
receptors and the receptors in muscles, tendons, and
joints |
A |
Mechanoreceptors |
1 |
Skin. Type I and type II receptors, G, hair receptors,
field receptors, 0 hair receptors, C mechanoreceptors,
pacinian corpuscle (PC) receptors, G1 hair receptors, SA
receptors, RA receptors |
2 |
Muscle and tendon. Muscle spindles, Golgi tendon organs,
pressure receptors, PC receptors
|
3 |
Joint. SA
type 1 receptors, SA type 2 receptors, . phasic" receptors,
"tap" receptors |
B |
4 |
Thermoreceptors. Warm and cold
receptors |
C |
5 |
Nociceptors. Pain
receptors |
II |
Special somatic
receptors. Respond to adequate stimulation of the organ
of Corti of the inner ear, the retina of the eye, and
the crista ampullaris and macula of the vestibular
system
|
A |
Mechanoreceptors. Organ of Corti hair cells and vestibular
system hair cells (type I and type
II) |
B |
Photoreceptors. Rods and cones of the retina |
III |
General visceral receptors. Respond to adequate stimulation of
the viscera and blood vessels |
A |
Mechanoreceptors. Carotid sinus and aortic baroceptors, alveolar
stretch receptors, GI stretch
receptors, urinary bladder stretch receptors B Thermoreceptors.
Warm and cold receptors |
C |
Nociceptors. Pain receptors |
D |
Chemoreceptors. Carotid body and aortic chemoreceptors
|
IV |
Special visceral receptors. Respond to adequate
stimulation of taste cells and the olfactory epithelium
|
A |
Chemoreceptors. Taste cells and olfactory cells |
|
 |
 |
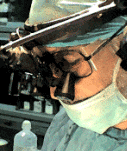
Prof. Munir Elias
Our brain is a mystery and to understand it, you
need to be a neurosurgeon, neuroanatomist and neurophysiologist.
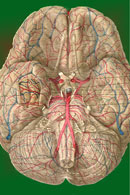
neurosurgery.tv

Please visit this site, where daily neurosurgical activities are going
on.
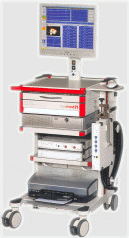
Inomed ISIS IOM System
|
|
|
|