SKELETAL MUSCLE CONTRACTION AND THE MOTOR UNIT
Most of the
important contributions to our current understanding of muscle
contraction and coordination have been made since the turn of
the twentieth century. Early observations utilizing the
sartorius muscle of the frog helped to demonstrate the
characteristics of the individual muscle twitch and also
established that contracting muscles produce heat and are
sensitive to the effects of temperature. Ultrastructural studies
of individual muscle fibers (cells) were just beginning at this
point. while the "sliding filament" theory describing muscle
contraction is just over 50 years old.
Researchers
have learned that muscle contraction cannot proceed in the
absence of adenosine triphosphate (ATP) and Ca2+
ions. Most of our assumptions about the role of these two
components during contraction is explained by the use of models.
Current models are most often based on the classic work of A. F.
Huxley, who in 1957 proposed a theory concerning the interaction
of the filaments actin and myosin in the contraction process of
skeletal muscle.
The
functional units of skeletal muscle are not individual muscle
fibers, but larger systems called motor units. The motor unit
consists of a motor neuron and the group of skeletal muscle
fibers which it innervates. An entire muscle may be composed of
thousands of such units representing millions of individual
muscle fibers
SKELETAL MUSCLE
CONTRACTION
A single
skeletal muscle is composed of many thousands to millions of
long, narrow contractile cells called muscle fibers (Fig-1).
These fibers are clustered together in parallel bundles called
fasciculi. Each muscle fiber is 10 to 80
µm in diameter and is
composed of hundreds to thousands of even smaller units called
myofibrils. Myofibrils contain the proteins actin and myosin,
which are the sliding
myofilaments
that are activated during
muscle contraction.
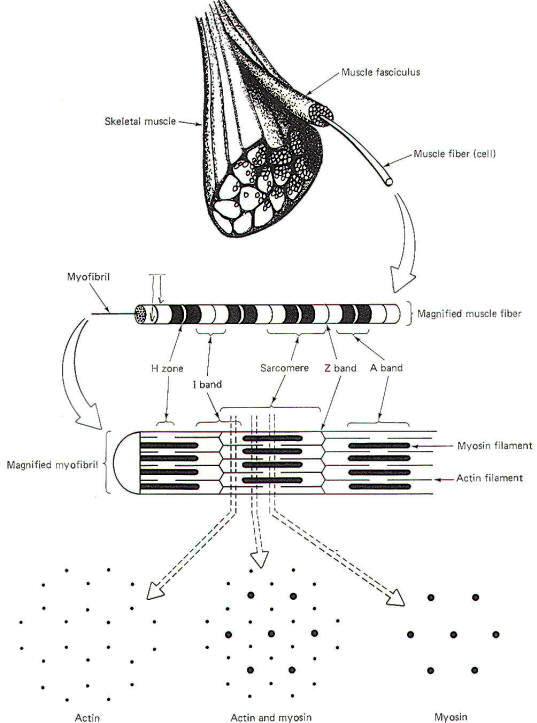 |
Fig-1 |
Each
individual muscle fiber is innervated by a single branch from a
motor neuron. This branch (telodendron) forms a neuromuscular
junction (NMJ) with the muscle cell membrane (sarcolemma),
Impulses arriving on the nerve fiber are transmitted to the
sarcolemma and ultimately cause the contraction of the muscle
fiber. A muscle fiber is a multinucleated cell whose sarcoplasm
(cytoplasm) contains mitochondria and stores of glycogen. The
availability of glycogen, which is easily converted to glucose,
ensures that the mitochondria will have sufficient amounts of
this readily available nutrient as an energy source for the
synthesis of ATP, a high-energy phosphate molecule needed to
energize the contractile process. In times of low muscle
activity, excess ATP is temporarily converted to creatine
phosphate.
Most of the
millions of individual muscle fibers within a single muscle run
the entire length of the muscle. Because they run parallel to
each other, the tensions developed by the individually
contracting fibers summate to produce the overall tension
developed by the muscle. In a sustained contraction, the
individual muscle fibers alternate firing with each other so
that some are contracting while others are relaxing. This
process helps avoid fatigue yet maintains a smooth and prolonged
muscle contraction.
Individual
myofibrils present a striated appearance of alternating light
and dark bands (Fig-1). The wide dark bands (A bands) represent
the region of relatively thick parallel-running myosin
filaments. The white bands (I bands) represent the region of
parallel-running actin filaments. The I band is bisected by a
thin dark zone, the Z line. A narrow light region (H zone)
bisects the A band. This distance between two Z lines is a
sarcomere, typically 2 µm
long in the resting muscle fiber.
During
contraction, opposing actin filaments slide toward each other
over the myosin, shortening the sarcomere and causing a
narrowing of the I band. Because the bands and lines of each of
the thousands of parallel myofibrils within a muscle fiber are
adjacent to each other, the banded appearance is also
characteristic of the entire muscle fiber.
Calcium Release by the Longitudinal
Sarcoplasmic Reticulum (LSR)
The
arrival of impulses at the end plate of the motor neuron causes
the release of ACh into the synaptic cleft between the end plate
and the folded muscle fiber membrane. This typically produces an
end plate potential (EPP) in excess of the excitation threshold,
generating impulses which travel over the muscle fiber membrane
and ultimately deep into the muscle fiber and activating the
contractile process. Extracellular fluid-filled channels called
T tubules travel through the muscle fiber at right angles to the
surface (Fig-2). In humans these channels typically traverse
that part of the muscle fiber where actin and myosin overlap.
Among the myofibrils between the T tubules, are Ca2+rich
organelles known as the longitudinal sarcoplasmic reticula
(LSR). The cisternae (enlarged ends of the LSR near the T
tubules) are particularly rich in Ca2+ ions (Fig-2).
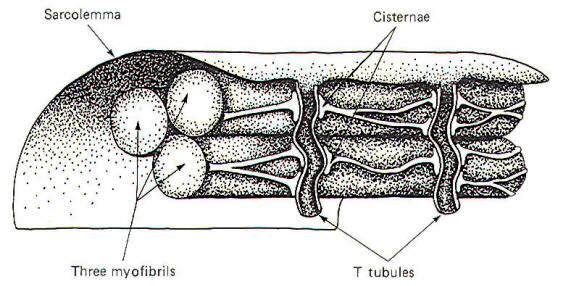 |
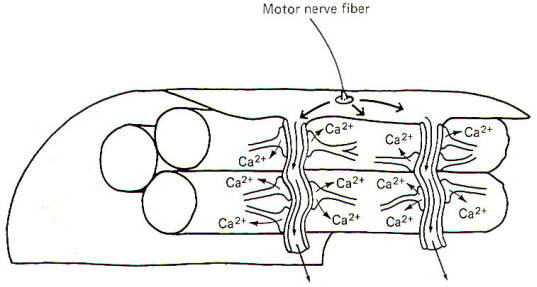 |
Fig-2 |
Fig-3 |
When
impulses are generated on the sarcolemma, they travel over its
surface and down the T tubule (Fig-3). The arrival of the
impulse in the vicinity of the cisternae causes the sudden
(within microseconds) release of large quantities
of Ca2+ ions into the sarcoplasm where the actin and
myosin overlap. These free Ca2+ ions then contribute
toward activating armlike extensions of the myosin filaments,
known as cross-bridges, which subsequently attach to the actin
filaments and slide them inward toward the center of the
sarcomere, causing the muscle fiber to shorten. As long as the
Ca2+ remains in the sarcoplasm, the muscle fiber will
remain contracted. Once impulses stop traveling across the
sarcolemma, the Ca2+ is immediately and actively
reabsorbed back into the cisternae and the muscle fiber relaxes.
Myosin Filaments
Each myosin
filament is composed of approximately 200 myosin molecules, each
of which has a molecular weight of 450,000. Each molecule has a
light meromyosin shaft and a heavy meromyosin armlike extension,
the cross-bridge (Fig-4). The shaft is formed by two twisted
strands of polypeptide which are more or less continuous with
two twisted strands in the cross-bridge arm. At the tip of the
arm is a head composed of globular protein. The heavy meromyosin
of the arm and head form the cross-bridge. The cross-bridge is
hinged to allow movement between the head and the arm and again
between the arm and the shaft.
The shafts
of approximately 100 myosin molecules lie together in an orderly
fashion at each end of the myosin filament. Approximately 50
crossbridge pairs radiate out from the central axis of the
myosin filament at each end. The myosin filament is about 1.6
µm long with cross-bridges
radiating out from most of its length with the exception of a
small region (0.2 µm) at its
equatorial point.
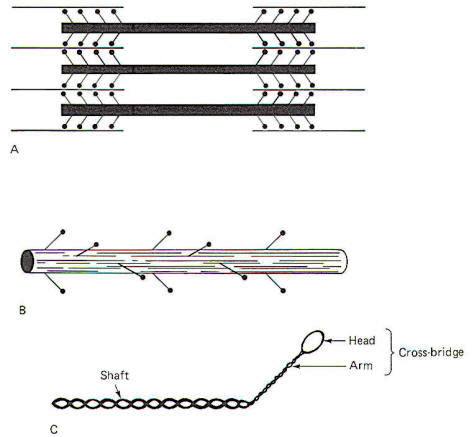 |
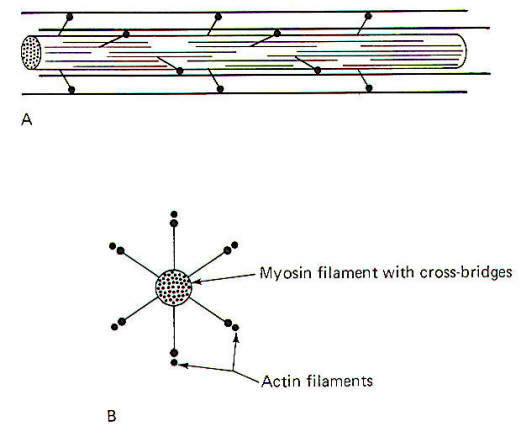 |
Fig-4 |
Fig-5 |
The
radiation of cross-bridge pairs is regular and orderly with each
crossbridge emerging 14.3 nm from the previous pair in the
filament. In addition, each cross-bridge pair is displaced
axially 1200 from the previous pair. Thus every third
pair is in the same spatial plane and is separated by a linear
distance of 42.9 nm. Because of this spatial arrangement, six
helically arranged actin filaments can make multiple contacts
with the cross-bridges at each end of the myosin filament
(Fig-5).
Actin Filaments
The actin
filament is composed of two kinds of actin. These are G actin
and F actin. G actin is composed of small protein molecules
(molecular weight, 47,000) capped by a molecule of adenosine
diphosphate (ADP). This unit complex, about 5.4 nm in length, is
polymerized to form a long strand of F actin. An actin filament
is formed when two F actin strands are helically twisted
together around tropomyosin, which lies in the groove between
the two. Troponin, associated with the tropomyosin,
configurationally "covers" the ADP sites of the individual G
actin molecules when the muscle fiber is relaxed (Fig-6). The
ADP sites occurring every 2.7 nm along the actin filament are
the active sites to which the heads of the myosin cross-bridges
attach. In the resting muscle no attachments are made as the
troponin effectively prevents interaction of the two. However,
when the muscle fiber is stimulated and Ca2+ ions are
released by the cisternae, the troponin (which has a high
affinity for Ca2+ ions) binds with them and is
configurationally reoriented so as to uncover the active ADP
sites, allowing the heads of the myosin cross-bridges to bind.
 |
Fig-6 |
The Contractile Mechanism
In the
resting state, the actin and myosin are not in contact because
of the interference of the troponin. Thus the sarcomere is at
its relaxed 2-µm length. The
heads of the cross-bridges are in a "cocked" state, storing
potential energy. When the cocked heads bind with the ADP active
sites (following Ca2+ release), some unknown trigger uncocks the
heads, causing them to pivot at their hinges with the arms and
sliding the actin filaments inward. The entire arm also pivots
slightly (Fig-7).
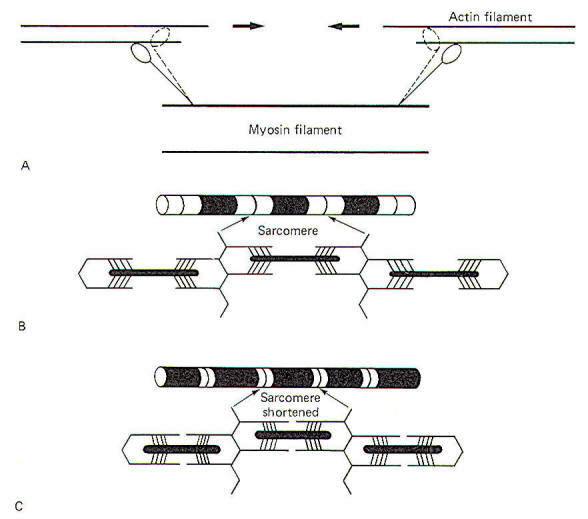 |
Fig-7 |
Sarcoplasmic ATP causes the heads of the cross-bridges to let go
of the actin filaments and provides the energy for recocking
them. The head of the cross-bridge itself probably provides the
adenosine triphosphatase (ATPase) activity for this process.
Subsequently the recocked heads bind with other active sites,
uncocking them and sliding the actin filaments still further
along, and so on. Consequently the sarcomere is shortened with a
noticeable decrease in the width of the I band.
THE MOTOR UNIT
Motor
unit consists of a motor neuron and the group of skeletal muscle
fibers which it innervates. Three types of motor units are found
in skeletal muscle. The largest of these are the type A motor
units, which are characterized by high contractile speed and
power. The term largely refers to the relative number of muscle
fibers in the motor unit. Type B motor units are the smallest
and are characterized by slow contractile speed and relatively
little power, but a high resistance to fatigue. Type C motor
units seem to represent a compromise between the other two. They
are intermediate in size, contractile speed and power, and
susceptibility to fatigue. These and other characteristics of
the three types of motor units are listed in Table-1.
Table-1
Characteristics of Motor Unit Types |
Characteristic
|
Type A
|
Type B |
Type C |
Size of motor unit
|
Large
|
Small |
Intermediate
|
Size of muscle
fiber |
Large |
Intermediate
|
Small |
Type of muscle
fiber |
A |
B |
C |
Contraction speed |
Fast |
Slow |
Intermediate |
Contraction
tension |
High |
Low
|
Intermediate |
Tetanization
frequency |
High |
Low
|
Intermediate |
Maximum tetanic
tension |
High |
Low
|
Intermediate |
Myoglobin
concentration |
Low
|
Intermediate |
High |
Glycogen
concentration |
High |
Intermediate |
Low |
Mitochondrial
ATPase |
Low
|
Intermediate |
High |
Capillary supply |
Low
|
Intermediate |
High |
Resistance to
fatigue |
Low
|
High |
Intermediate |
The
specific contraction requirements of a particular muscle
determine the type of motor units found in that muscle. Muscles
which must produce great tension but are only called on
periodically will likely incorporate a high percentage of type A
motor units in their organization. Such muscles trade off
resistance to fatigue in favor of contractile speed and power.
On the other hand, muscles which must support the body against
gravity in maintaining the upright posture must be continually
active and demonstrate a high resistance to fatigue. Such
muscles would be expected to incorporate a high percentage of
type B units in their design. Still other muscles need to
incorporate the best features of both and include a percentage
of type C units along with the others.
A single
muscle often contains all three types of motor units.
Nevertheless, limb muscles often show a preponderance of type A
or type B units and are thus often classified as "fast" (phasic)
or "slow" (tonic) muscles, respectively. The gastrocnemius is an
example of the former, while the soleus is an example of the
latter. In order to appreciate the characteristics of each type
of motor unit, let's compare the contractile characteristics of
these two muscles.
Properties of the Soleus and
Gastrocnemius Muscles of the Cat
The soleus
and gastrocnemius muscles are well suited for comparison. While
each has a different origin, they insert together into the
common tendon of the calcaneus and serve to extend the foot.
Nevertheless, their histology and contractile characteristics
are quite different, reflecting the tonic role of the soleus in
providing continual support of the body against gravity and the
more transient role of the gastrocnemius in powering the phasic
activities of walking, running, and jumping.
The soleus
is a good example of a slow-twitch tonic muscle. Its fibers must
be continually active while a person is standing in order to
give support against gravity. It plays a similar role in the
cat. Consequently it must be resistant to fatigue.
Appropriately we find that its fibers contain a large amount of
mitochondria, enabling it to easily produce the large amounts
of ATP needed to power its continual contractions. Similarly
its fibers are amply supplied with capillaries able to saturate
the oxygen-carrying pigment myoglobin. which is abundantly
found in its type B muscle fibers. This is a necessary feature
for the aerobic production of ATP by its mitochondria. The red
color of the soleus and other such muscles is due to the color
of the myoglobin as well as the blood in the muscle's abundant
capillary supply.
Pale
muscles such as the gastrocnemius are often noted for periodic
strong contractions rather than continual use. They are
characterized by larger sarcoplasmic reticula than are found in
red muscles such as the soleus. This enables them to release
large amounts of Ca+2 quickly, producing rapid and strong
contractions. Because such muscles lack large amounts of myoglobin, mitochondria, and extensive capillary supplies,
their ability to aerobically produce ATP after a period of
strong activity is considerably less than that of most red
muscles. Hence they are also more susceptible to fatigue. The
correlation between color and speed of contraction is not always
perfect. however, it should be cautious about
thinking of red muscle as being synonymous with slow twitch and
pale muscles with fast twitch.
Types of Muscle Fibers
Like motor
units, muscle fibers are also classified by type. When muscles
are specifically treated to assay them quantitatively for
mitochondrial ATPase, three types of fibers can be identified.
The largest of these contain relatively few mitochondria, are
poorly supplied with capillaries, show little mitochondrial
ATPase, contain relatively little myoglobin, and are pale in
color. These are type A muscle fibers. They correspond to type A
motor units. Type C muscle fibers represent the opposite
extreme. They are the smallest fibers, contain the highest
amount of myoglobin, are dark in color, are amply supplied with
capillaries, contain many mitochondria, and show the highest
ATPase activity. They correspond to type C motor units. Type B
muscle fibers are intermediate in size, mitochondrial
concentration, ATPase activity, capillary supply, and myoglobin
concentration. They correspond to type B motor units.
The soleus
is composed almost exclusively of type B fibers. The
gastrocnemius, on the other hand, contains all three types;
however, type A fibers constitute about 50 percent of the fiber
population, and because of their relatively large size actually
make up about 70 percent of the bulk of the muscle. The rest is
composed of type B and type C fibers.
Size and Firing Rate of Motor Unit
Neurons
Certain
characteristics of motor units are determined by the properties
inherent in the motor neuron itself. The motor units in the
soleus muscle are innervated by small, slowly conducting alpha
motor neurons. On the other hand, the neurons which innervate
the large type A- muscle fibers of the gastrocnemius muscle are
larger and have greater conduction velocities.
The size of
the neuron cell body is directly related to the diameter of the
conducting fiber. Small-diameter nerve fibers have small cell
bodies. Experimentation has shown that the smaller the cell
body, the lower the excitation threshold for the production of
an action potential. Therefore, the excitability of a neuron is
an inverse function of its size, and less input stimulation is
subsequently required to fire it. Therefore the participation
of a motor unit in a graded muscle activity is dictated by the
size of its motor neuron. Now recall that if the firing rate of
a neuron depends on the degree to which its central excitatory
state (CES) exceeds the excitation threshold (ET), it is not
surprising to find that a CES of 30 mV would produce a higher
firing rate in a small motor neuron with a low excitation
threshold than it would in a larger neuron with a higher
threshold (Fig-8).
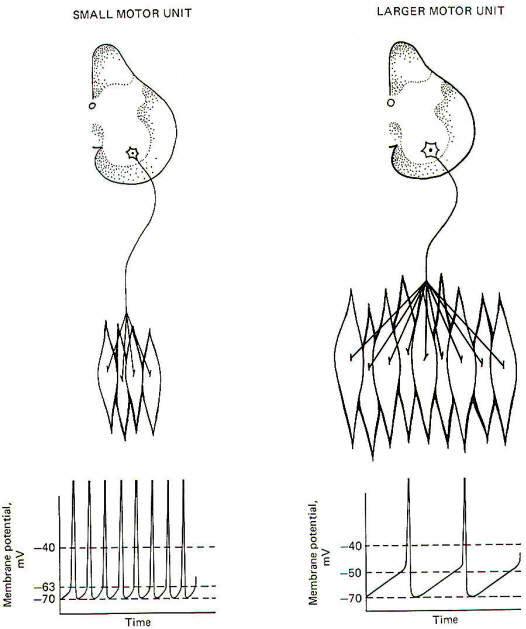 |
Fig-8 |
The number
of muscle fibers in a motor unit is also directly related to the
size of its motor neuron. Small motor nerve fibers form small
motor units and large motor nerve fibers form large motor units.
Since small motor neurons fire more frequently than larger
neurons because of their relatively greater susceptibility to
discharge, it follows that the muscle fibers in these small
motor units are more heavily "used" than those associated with
larger units. Because of this high firing rate, small motor
units must be relatively resistant to fatigue. Therefore it is
not surprising to find their overwhelming incorporation into
muscles which are often continually active and require high
fatigue resistance such as the soleus.
Conversely
the gastrocnemius, a phasic muscle, is subject to intermittent
bursts of high activity. Its motor units have higher excitation
thresholds because of the relatively larger motor neurons
innervating its type A muscle fibers. These units will become
active only when the input stimulation to the motor neuron pool
in the spinal cord reaches a sufficiently high level.
Nevertheless, the resting muscle tone found in the
gastrocnemius and other such muscles is probably due to the
activity in its type B and C motor units, which are more
susceptible to firing and thus maintain a steady discharge
frequency. Any long-term resistance to fatigue which these
muscles possess is also probably due to the activity in its type
Band C motor units. There is much evidence that world class
long-distance runners have a higher than normal percentage of
type Band C motor units in their phasic muscles, enabling them
to cover many miles of continuous running without significant
muscle fatigue.
Contractile Tension
Large motor
units produce more tension than smaller motor units. This is
possible because the large units incorporate more muscle fibers
than small units. We also know that motor units obey the
all-or-none principle, which means that if the motor unit fires
at all, all of its muscle fibers contract together. Now because
all the fibers in a muscle run parallel to each other, the
tension produced by each is added to all the others, producing
the combined tension of the motor unit. Examination of Fig-9
will show that the contractile tension developed by the motor
units within a single muscle are not identical. Instead they
represent a wide range which gives the muscle a choice of
variable tension which it would not otherwise possess.
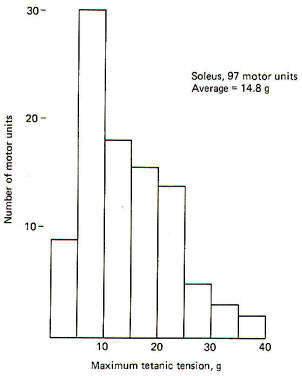 |
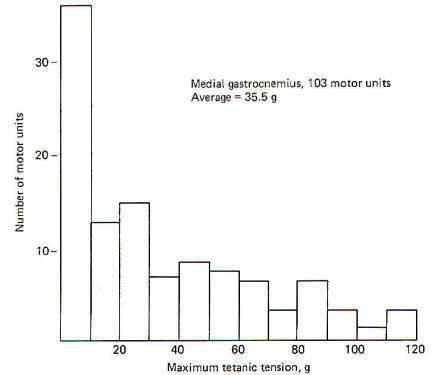 |
Fig-9 |
Fig-10 |
The maximal
tetanic tension of a representative sample of 97 motor units of
the soleus muscle are plotted in 5-g groups against the number
of units in each group. To obtain the records, a stimulating
current was delivered to 97 individual motor nerve fibers in
the ventral root of the VIIth lumbar and 1st sacral nerves of
the cat. Muscle tension was measured by connecting the soleus
muscle in series with a transducer.
Notice that
the maximum tension developed by the largest motor units of the
soleus was 40 g. The average tension was 14.8 g per unit.
Compare this with the higher tension developed by 103
representative motor units from the gastrocnemius muscle when it
was similarly examined (Fig-10). In this case the motor units
are plotted in 10-g groups against the number of units in each
group. As might be expected from the relatively large motor
units found in the gastrocnemius muscle, the average tension per
unit is higher (35 g per unit) with its largest units producing
up to 120 g.
Contractile Speed
Certain
characteristics of the motor unit are functions of qualities
inherent in the muscle fibers themselves. Nevertheless, the
different qualities possessed by muscle fibers are also
determined to some extent by the type of nerve fibers which
innervate them. During fetal development, at the time of their
first innervation, all the limb muscle fibers in mammals are
similar in contractile behavior. However, following
innervation, each motor unit develops a speed of contraction
which is determined by its motor neuron. Fast-twitch muscle
fibers are innervated by the large motor neurons, while
slow-twitch muscle fibers are innervated by smaller motor
neurons.
There seems
to be little doubt that the neuron exerts a trophic influence on
the development of the muscle fiber. In a telling experiment
with 1-day-old kittens, J. C. Eccles showed that the type of
motor innervation determines to some extent the speed of muscle
contraction which develops. He separated the nerve to one
fast-twitch and one slow-twitch muscle of the hind leg. He then
reconnected the nerve portion which formerly innervated the
slow-twitch muscle to the fast-twitch muscle. He similarly
reconnected the nerve portion formerly innervating the
fast-twitch muscle to the slow-twitch muscle. After reinnervation had been successfully completed and the kitten had
recovered, he noted that the former fast-twitch muscle now
contracted more slowly while the former slow-twitch muscle now
contracted more quickly. Evidence now indicates that changes in
twitch velocity following such reinnervation experiments
probably results from alteration in the ATPase activity of the
myosin and the rate of Ca2+ ion release by the cisternae of the
LSR. Examination
of Fig-11 will show that the soleus is a slow-twitch muscle.
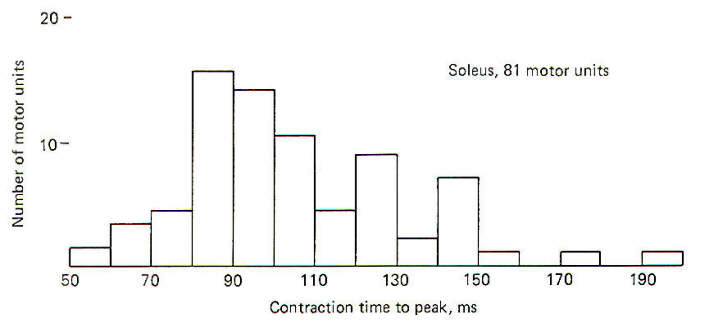 |
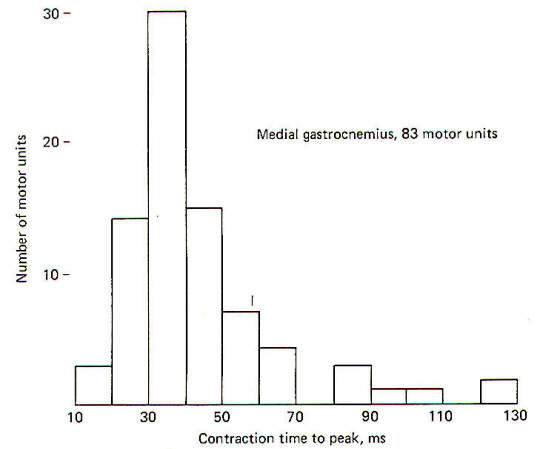 |
Fig-11 |
Fig-12 |
When the
time to the peak of contraction of 81 randomly selected motor
units is plotted against the number of units in each 10-ms
group, we see that there is a wide range of contraction times
within the muscle. The shortest time is 58 ms and the longest is
193 ms, with the greatest number falling between 80 and 90 ms.
The
slow-twitch nature of the soleus muscle can be seen when it is
compared to the gastrocnemius. When the contraction times of 83
randomly selected gastrocnemius motor units were plotted against
the number of units in each 10-ms group. it was observed that
they fall into two groups: a large one from 18 to 70 ms and a
smaller one from 84 to 129 ms (Fig. 5-12).
There is a
relationship between the contraction velocity and the tension
developed by a motor unit. As a group, large motor units (those
producing the most tension and innervating the greatest number
of muscle fibers) contract quickly, while smaller motor units
produce less tension and contract more slowly.
Stimulating Frequency Required for
Tetanization
If a
contracting muscle is stimulated again before it has had a
chance to fully relax, a second contraction will fuse with the
first, producing tetanus. The minimum stimulating frequency
necessary to do this depends on the duration of the previous
twitch (single contraction in response to a single stimulus).
Motor units with brief contraction times (larger units) require
a higher stimulating frequency to produce tetanus than do
smaller slow-twitch units. In Fig-13 a large and small motor
unit from the gastrocnemius of the cat were stimulated
repeatedly at 5, 10, 20,50, and 100 stimuli per second. Notice
that the large unit in column A showed little tetanus until the
frequency reached 20 per second and didn't develop maximum
tension until the frequency reached 100 per second. By
comparison, the small motor unit in column B began to tetanize
at the relatively low frequency of 10 per second and was nearly
maximal at 20 per second. Column C shows the response of a
soleus motor unit similar in size to the small gastrocnemius
motor unit in column B. Remember that most of the motor units in
the gastrocnemius muscle have shorter contraction times than
most of the soleus units. It is not surprising to find that the
average frequency required for tetanization of the gastrocnemius
motor units is greater than we find in the soleus motor units.
Nevertheless, the gastrocnemius does contain some small motor
units with contraction speeds and tetanization frequencies
similar to small units in the soleus. These two are compared in
columns Band C in Fig-13.
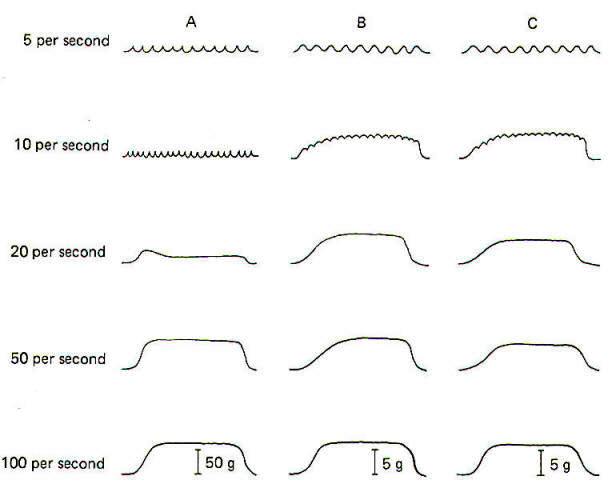 |
Fig-13 |
Maximum Tetanic Tension
A second
examination of Fig-13 will show that the total tetanic
tension developed by the large soleus motor unit in column A is
nearly 8 times greater than that developed in the smaller unit
of column B. Once again, however, because the gastrocnemius is
primarily made up of motor units which are larger than those
found in the soleus, the maximum tetanic tension developed by
its motor units is typically larger.
The maximum tetanic tensions developed by the two motor units in
column B and C are identical because the size of the motor units
is identical.
Further
examination of Fig-13 will show that the total tension
developed during a tetanic contraction is greater than that
developed during a single twitch. The reason for this is not
known, but may be due to a less-than-maximum amount of Ca 2+
release by the LSR during a single twitch. It may require
several consecutive twitches to release enough Ca 2+ to activate
all of the crossbridges and produce maximum tension. The ratio
between the twitch tension and the maximum tetanic tension is
between 0.2 and 0.25 for both slow- and fast-twitch muscles.
Resistance to Fatigue
An ideal
muscle would be able to develop great tension when needed. doing
it quickly and smoothly. In addition. it would be able to
maintain a high level of activity for prolonged periods of time
without fatiguing. Actual muscles exhibit some of these
characteristics, but not all of them. Therefore a
good compromise all-purpose muscle is one which contains
different types of motor units, each capable of producing one or
more of the desired characteristics. As previously noted.
muscles which of necessity are used intensely for prolonged
periods of time are generally composed of small motor units
innervating small muscle fibers rich in myoglobin, mitochondrial
ATPase. and capillary supply. They contract slowly and produce
minimum tension but can operate for extended periods of time
without fatiguing because of their ability to produce large
amounts of ATP. Recall also that motor units of such muscles
are heavily "used" because of their low excitation thresholds,
which produce high firing rates in their motor neurons. Such
muscles have clearly had to compromise between contractile
speed and power and the need to resist fatigue, choosing the
latter as the more important feature for their particular role.
The soleus is such a muscle.
Since not
all muscles of the body are exclusively involved in tonic or
phasic activity, it is not surprising to find that most muscles
are a heterogeneous mixture of all three types of motor units,
varying the ratio between the types of units in order to achieve
the best possible compromise of contractile characteristics
suited for their particular range of activities.
Innervation Ratio and Fine Control
A single
motor nerve fiber can innervate any number of muscle fibers from
one up to several thousand. The innervation ratio represents the
number of muscle fibers innervated by a single motor nerve
fiber. A small motor unit might have an innervation ratio as low
as 10:1. Some of the large motor units of the gastrocnemius
have been estimated to be as high as 2000:1. The innervation
ratio of its motor units confers certain qualities to a muscle,
as we have already seen.
An
additional quality not previously examined is the smoothness
with which fine increases in tension can be added to a
contracting muscle. Muscles primarily composed of small motor
units are capable of finer, more gradual changes in contractile
tension and thus are capable of finer movements than muscles
composed primarily of larger motor units. For example, certain
muscles of the fingers have innervation ratios as low as 10:1.
This means that if a slight increase in tension is called for in
order to perform a certain delicate task, the recruitment of one
more motor unit will add the tension of only 10 more muscle
fibers. This allows for very fine and controlled increments in
tension. This is a very important feature in muscles which are
often called upon to perform fine delicate and controlled
movements. The trade-off which these muscles make in gaining
fine control is the lack of contractile speed and power,
features which aren't that important in such muscles anyway.
Compare
this with the gastrocnemius muscle of the calf whose largest
motor units have innervation ratios as high as 2000:1.
Obviously, firing one more motor unit in this muscle adds the
combined tension produced by 2000 additional muscle fibers. This
obviously increases the overall tension of the muscle but
certainly by a less finely controlled increment than in finger
muscles. Of course the ability to add large amounts of tension
quickly is obviously more important in the gastrocnemius than
are finely graded increments of low tension.
Order of Motor Unit Recruitment during
a Progressing Muscle Contraction
As a motor
act proceeds from little to maximum strength, motor units with
precise characteristics are progressively recruited in a logical
order. First are the smallest tonic motor units, followed by
larger tonic units, and finally by the largest tonic units. Now
if the motor act requires fine control only and not a great deal
of tension. the recruitment of motor units might stop here.
However, if strength is also required, the higher-threshold
phasic units are recruited next. Depending on how much strength
is required for the particular motor act, appropriate numbers
and types of additional phasic motor units will be recruited.
Again, the order will be the smallest phasic units (those with
the lowest thresholds) followed by larger and finally the
largest phasic units.
Recognize
that tonic motor units, because of their low tetanization
frequencies, can alternate firing to give finely controlled yet
long-lasting and smooth contractions at low tension. Thus
because of their relatively long twitch durations, some tonic
units can begin to relax while others begin to contract while
continuing a smoothly maintained level of muscle tension. Phasic
units, on the other hand, lack fine control in sustaining smooth
contractions because of their short twitch durations, which make
tetanus, and hence a smooth alteration of motor unit firings,
less likely.
Factors Determining the Final Strength
of Contraction
The
final-strength of any muscle contraction is determined by two
factors. The first is the firing rate of the motor units
involved, while the second relates to the number and types of
units incorporated in the contraction. We have already seen that
increasing the firing rate of an individual motor unit will
increase the final strength of contraction. Recall that the
maximum tetanic tension of a motor unit is considerably greater
than the tension produced by a single twitch (Fig-13). It is
important to recognize that tetanus in this case is a normal and
certainly desirable physiological event adding progressively to
the tension developed by the motor unit. We should also
recognize that the recruitment of additional motor units adds
to the final strength of contraction. Also, because phasic motor
units develop higher tension than tonic units, the final
strength is partially a function of which type is employed.
|